Ribosomes are essential, tiny, granular organelles present in both eukaryotic and prokaryotic cells. These remarkable structures are found within the cytosol of the cell and are primarily responsible for synthesizing proteins, which are vital for various cellular functions. The process of protein synthesis involves translating genetic information carried by messenger RNA (mRNA) into functional proteins that execute countless tasks within living organisms. Understanding ribosomes’ structure, function, and significance offers a deeper insight into the fundamental processes that sustain life.
Table of Contents
Ribosome Definition
A ribosome is a complex cellular structure that assembles proteins by linking together amino acids according to genetic instructions conveyed by mRNA. This definition encapsulates the core function of ribosomes within cells, highlighting their role as molecular machines that execute protein synthesis, also known as translation.
Discovery and Historical Context
The study of ribosomes began with the observations of George Palade in 1953. Utilizing an electron microscope, Palade identified these granular structures within cells, marking a significant advancement in our understanding of cellular biology. His discovery laid the groundwork for future research into ribosomes’ role in protein synthesis, ultimately earning him a Nobel Prize in 1974 for his contributions to cell biology.
Ribosome Structure
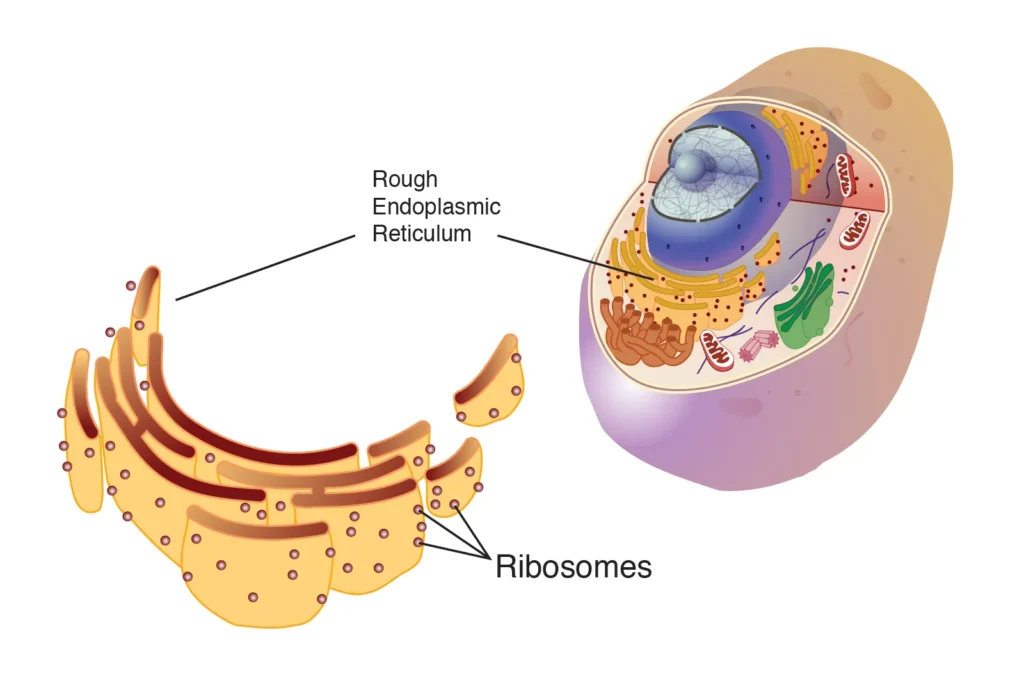
Overview of Ribosomal Composition
Ribosomes are composed of two distinct subunits: one large and one small. These subunits are each made up of proteins and ribosomal RNA (rRNA) molecules, which together form a functional ribosome. The structural complexity of ribosomes reflects their essential role in accurately translating genetic information into proteins.
- Small Subunit: The small subunit is responsible for reading the genetic information encoded in the mRNA. This subunit binds to the mRNA strand and ensures that the sequence is read correctly during the process of translation.
- Large Subunit: The large subunit plays a crucial role in catalyzing the formation of peptide bonds between amino acids. These bonds link the amino acids together, forming a growing polypeptide chain that eventually folds into a functional protein.
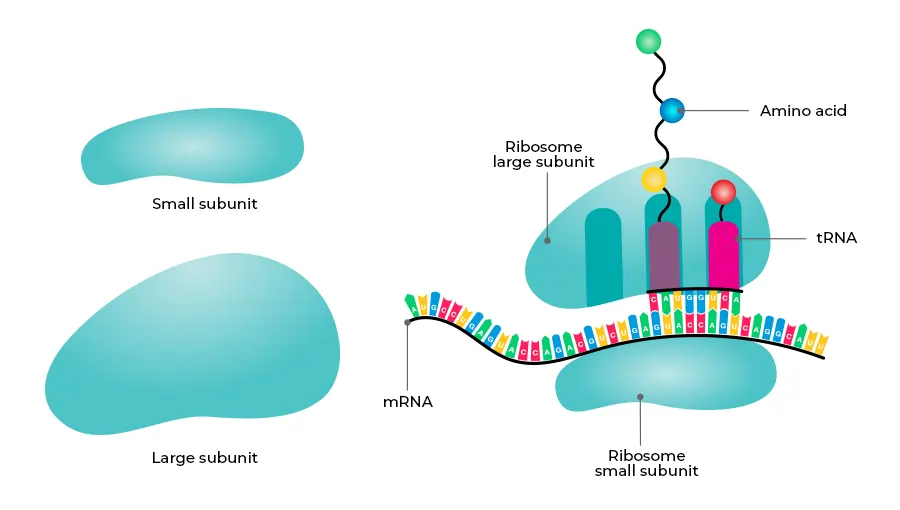
Specific Binding Sites
Ribosomes possess specific binding sites for different molecules involved in protein synthesis. These sites include:
- A (Aminoacyl) Site: This site is where aminoacylated tRNA molecules are accepted during translation. The aminoacyl-tRNA brings the appropriate amino acid to the ribosome, which is then added to the growing peptide chain.
- P (Peptidyl) Site: The P site holds the tRNA carrying the growing peptide chain. As new amino acids are added, the peptide chain is transferred to the tRNA at this site, enabling the continuation of protein synthesis.
- E (Exit) Site: After the amino acid has been added to the peptide chain, the tRNA molecule moves to the E site, where it is deacylated and eventually leaves the ribosome.
Types of Ribosomes: Free and Bound
Ribosomes can be classified into two types based on their location within the cell:
- Free Ribosomes: These ribosomes are found floating freely within the cytosol. They typically synthesize proteins that function within the cytosol or are transported to organelles such as the mitochondria or nucleus.
- Bound Ribosomes: Bound ribosomes are attached to the endoplasmic reticulum (ER), forming what is known as the rough endoplasmic reticulum (RER). These ribosomes produce proteins that are destined for secretion outside the cell, incorporation into the cell membrane, or use within lysosomes.
Ribosomal Subunits: A Comparison Between Prokaryotes and Eukaryotes
The structure of ribosomal subunits differs between prokaryotic and eukaryotic organisms:
- Prokaryotic Ribosomes (70S): Prokaryotes, such as bacteria, have 70S ribosomes, which consist of a small 30S subunit and a large 50S subunit. The “S” stands for the Svedberg unit, a measure of the rate of sedimentation during centrifugation, which reflects the size and density of the ribosomal subunits.
- Eukaryotic Ribosomes (80S): Eukaryotes, including plants, animals, and fungi, possess 80S ribosomes. These ribosomes are larger and more complex, with a small 40S subunit and a large 60S subunit.
The difference in ribosome size and composition between prokaryotes and eukaryotes is significant, as it allows for the development of antibiotics that specifically target bacterial ribosomes without affecting eukaryotic ribosomes. For example, antibiotics like tetracyclines and aminoglycosides exploit this difference to inhibit bacterial protein synthesis, making them effective treatments for bacterial infections.
Ribosome Location in the Cell
Distribution and Function
Ribosomes are distributed throughout the cell, either as free ribosomes within the cytosol or as bound ribosomes attached to the rough endoplasmic reticulum. This distribution allows ribosomes to fulfill various cellular functions by producing proteins needed in different locations.
In animal cells, there can be as many as 10 million ribosomes, reflecting the high demand for protein synthesis required to maintain cellular functions. Ribosomes often work together, with multiple ribosomes binding to a single mRNA strand to form a structure known as a polysome or polyribosome. This arrangement increases the efficiency of protein synthesis, allowing for the rapid production of multiple copies of a protein from a single mRNA molecule.
Rough Endoplasmic Reticulum and Ribosome Function
When ribosomes are attached to the endoplasmic reticulum, they contribute to the formation of the rough endoplasmic reticulum (RER). The RER is characterized by its studded appearance, which is due to the presence of ribosomes on its surface. The RER plays a critical role in the synthesis of proteins destined for secretion or incorporation into cellular membranes.
Protein Targeting and Transport
Proteins synthesized by ribosomes on the RER are often transported to the Golgi apparatus for further modification and sorting. The Golgi apparatus adds sugar molecules to the proteins, a process known as glycosylation, and packages them into vesicles for transport to their final destinations, such as the cell membrane or outside the cell.
Functions of Ribosomes
Protein Synthesis: The Central Role of Ribosomes
Ribosomes have two principal functions: decoding genetic messages and forming peptide bonds. These functions are essential for the production of proteins, which carry out numerous tasks within the cell.
Decoding Genetic Messages
During protein synthesis, ribosomes decode the genetic information carried by mRNA. The mRNA is transcribed from DNA in the nucleus and then transported to the cytoplasm, where ribosomes translate the mRNA sequence into a specific sequence of amino acids. This process involves the initiation, elongation, and termination stages of translation.
- Initiation: The small ribosomal subunit binds to the mRNA and locates the start codon (AUG), which signals the beginning of translation. The large subunit then joins the small subunit, forming a complete ribosome ready to synthesize a protein.
- Elongation: During elongation, aminoacyl-tRNA molecules bring specific amino acids to the ribosome, where they are added to the growing polypeptide chain. The ribosome moves along the mRNA, reading each codon and adding the corresponding amino acid.
- Termination: Translation continues until the ribosome encounters a stop codon (UAA, UAG, or UGA), signaling the end of the protein-coding sequence. The newly synthesized protein is then released from the ribosome, and the ribosomal subunits dissociate.
Peptide Bond Formation
Within the ribosome, rRNA catalyzes the formation of peptide bonds between amino acids. This reaction is known as the peptidyl transferase reaction. The peptide bonds link the amino acids together, forming a polypeptide chain that will eventually fold into a functional protein. The ribosome’s ability to catalyze this reaction is crucial for the efficient synthesis of proteins.
Protein Sorting and Distribution
After proteins are synthesized by ribosomes, they are sorted and distributed to various parts of the cell. Proteins produced by free ribosomes typically remain within the cytoplasm and are used in processes such as metabolism, cell signaling, and cytoskeletal maintenance.
In contrast, proteins synthesized by ribosomes bound to the RER are often transported to the Golgi apparatus for further modification. These proteins may be secreted outside the cell, incorporated into the cell membrane, or used within lysosomes for intracellular digestion.
Ribosome-Related Diseases
Ribosomopathies: Disorders of Ribosome Function
Disorders caused by the improper functioning of ribosomes are known as ribosomopathies. These conditions arise from mutations in the genes encoding ribosomal proteins or other components of the ribosome, leading to defects in ribosome biogenesis or function. Ribosomopathies often result in severe health problems, including bone marrow failure, anemia, and congenital syndromes.
Diamond-Blackfan Anemia (DBA)
Diamond-Blackfan anemia (DBA) is a rare blood disorder that affects the bone marrow, the tissue responsible for producing blood cells. In DBA, the bone marrow cannot produce enough red blood cells (RBCs), leading to anemia, a condition characterized by a lack of sufficient RBC
s to carry oxygen throughout the body.
DBA is associated with mutations in one of several genes encoding ribosomal proteins, resulting in defective ribosome function. These mutations cause abnormal pre-rRNA maturation, leading to impaired ribosome biogenesis and a reduction in the number of functional ribosomes within the cell.
X-Linked Dyskeratosis Congenita (DKC)
X-linked dyskeratosis congenita (DKC) is another ribosomopathy characterized by a wide range of symptoms, including skin abnormalities, nail dystrophy, and bone marrow failure. DKC is caused by mutations in the DKC1 gene, which encodes a protein involved in ribosome biogenesis and telomere maintenance. The resulting ribosomal defects contribute to the development of the disease.
Treacher Collins Syndrome (TCS)
Treacher-Collins syndrome (TCS) is a congenital disorder that affects craniofacial development. It is caused by mutations in genes such as TCOF1, which encodes a protein involved in ribosome biogenesis. The mutations lead to defects in ribosome function, particularly in neural crest cells, which are essential for craniofacial development. This results in the characteristic facial abnormalities associated with TCS.
Cartilage-Hair Hypoplasia (CHH)
Cartilage-hair hypoplasia (CHH) is a rare genetic disorder characterized by short stature, fine and sparse hair, and an increased risk of infections and certain types of cancer. CHH is caused by mutations in the RMRP gene, which encodes an RNA component of the mitochondrial ribonuclease complex involved in ribosome biogenesis. The resulting ribosomal defects contribute to the various symptoms of CHH.
Importance of Understanding Ribosomopathies
The study of ribosomopathies provides valuable insights into the fundamental processes of ribosome function and protein synthesis. Understanding these disorders can lead to the development of targeted therapies that address the underlying defects in ribosome biogenesis and function. For example, treatments for DBA may involve corticosteroids or hematopoietic stem cell transplantation, which can help restore normal blood cell production.
Key Informative Table About Ribosomes
The following table summarizes key information about ribosomes, including their structure, function, types, and related diseases. This table provides a concise overview of the critical aspects of ribosomes discussed in the article, offering a clear and organized way to understand the fundamental roles of ribosomes in cellular biology.
Category | Details |
---|---|
Definition | A cellular structure that assembles proteins by linking amino acids based on genetic instructions from mRNA. |
Discovery | Discovered by George Palade in 1953 using an electron microscope. |
Composition | Made of ribosomal RNA (rRNA) and proteins, consisting of two subunits: one large and one small. |
Subunits (Prokaryotes) | 70S ribosomes: Small 30S subunit and large 50S subunit. |
Subunits (Eukaryotes) | 80S ribosomes: Small 40S subunit and large 60S subunit. |
Binding Sites | A (Aminoacyl) Site: Accepts aminoacyl-tRNA. P (Peptidyl) Site: Holds the growing peptide chain. E (Exit) Site: Releases deacylated tRNA. |
Types | Free Ribosomes: Synthesize proteins used within the cytoplasm. Bound Ribosomes: Attached to the rough endoplasmic reticulum (RER) and synthesize proteins destined for secretion or membrane incorporation. |
Location | Found in the cytosol or attached to the rough endoplasmic reticulum (RER). |
Functions | Protein Synthesis: Decodes mRNA into proteins by linking amino acids. Peptidyl Transferase Reaction: Catalyzes the formation of peptide bonds. |
Associated Diseases | Ribosomopathies: Disorders due to ribosomal dysfunction, including Diamond-Blackfan anemia (DBA), X-linked dyskeratosis congenita (DKC), Treacher Collins syndrome (TCS), and Cartilage-hair hypoplasia (CHH). |
Importance | Essential for translating genetic information into functional proteins, maintaining cellular processes, and understanding related disorders. |
Conclusion
Ribosomes are essential organelles responsible for protein synthesis, a fundamental process that underpins the functioning of all living cells. By translating genetic information from mRNA into proteins, ribosomes enable cells to carry out a wide range of activities, from metabolism to cell signaling to maintaining structural integrity. The intricate structure and function of ribosomes, including their role in decoding genetic messages and forming peptide bonds, highlight their importance in cellular biology.
Moreover, the study of ribosome-related diseases, or ribosomopathies, sheds light on the critical role that ribosomes play in maintaining cellular health. Disorders such as Diamond-Blackfan anemia, X-linked dyskeratosis congenita, and Treacher-Collins syndrome illustrate the severe consequences of defective ribosome function, underscoring the need for continued research into ribosome biology and its implications for human health.
In conclusion, ribosomes are not only the site of protein synthesis but also the key to understanding many essential cellular processes and addressing disorders related to ribosomal dysfunction. As we continue to explore the complexities of ribosome structure and function, we gain deeper insights into the molecular machinery that sustains life.
Frequently Asked Questions (FAQs) About Ribosomes
What are ribosomes, and why are they important?
Ribosomes are complex molecular machines found in both prokaryotic and eukaryotic cells, responsible for synthesizing proteins by translating the genetic code carried by messenger RNA (mRNA). The importance of ribosomes cannot be overstated, as proteins are essential for nearly all cellular functions. Proteins act as enzymes to catalyze biochemical reactions, provide structural support, regulate cell signaling, and play key roles in immune responses. Without ribosomes, cells would be unable to produce the proteins necessary for survival and proper functioning, ultimately leading to cell death.
Ribosomes are composed of ribosomal RNA (rRNA) and proteins, forming two subunits: a large subunit and a small subunit. These subunits work together to ensure that amino acids are linked in the correct order, forming functional proteins. Given their central role in protein synthesis, ribosomes are often referred to as the “workbenches” of the cell.
How were ribosomes discovered, and who is credited with this discovery?
The discovery of ribosomes is credited to George Palade, a Romanian-American cell biologist who made significant contributions to the field of cell biology. In 1953, Palade observed tiny granular structures within the cytoplasm of cells using an electron microscope. These structures were later identified as ribosomes. Palade’s pioneering work in visualizing and describing ribosomes earned him a share of the Nobel Prize in Physiology or Medicine in 1974, alongside Albert Claude and Christian de Duve.
Palade’s discovery was groundbreaking, as it provided the first clear evidence of the cellular machinery involved in protein synthesis. This laid the foundation for future research into ribosomes and their critical role in translating genetic information into proteins. Today, Palade is remembered as one of the founding figures of modern cell biology, and his contributions continue to influence our understanding of cellular processes.
What is the structure of ribosomes, and how do they function?
Ribosomes have a unique structure that consists of two distinct subunits: a large subunit and a small subunit. Each subunit is composed of ribosomal RNA (rRNA) and ribosomal proteins. The small subunit is primarily responsible for reading the genetic information encoded in mRNA, while the large subunit catalyzes the formation of peptide bonds between amino acids, leading to the synthesis of proteins.
The process of protein synthesis begins when the small subunit binds to the mRNA. The large subunit then joins the small subunit, forming a complete ribosome. The ribosome moves along the mRNA strand, reading the sequence of codons, which are three nucleotide sequences that correspond to specific amino acids. Transfer RNA (tRNA) molecules, each carrying an amino acid, enter the ribosome at the A site. The ribosome facilitates the binding of the tRNA anticodon to the mRNA codon, ensuring that the correct amino acid is added to the growing peptide chain.
As the ribosome continues along the mRNA, the growing peptide chain is transferred to the tRNA at the P site. The newly formed peptide bond between amino acids is catalyzed by the rRNA in the large subunit. Finally, the empty tRNA exits the ribosome through the E site, and the process continues until the ribosome encounters a stop codon, signaling the end of translation.
What are the differences between prokaryotic and eukaryotic ribosomes?
Prokaryotic and eukaryotic ribosomes differ in size, composition, and sedimentation rate, as measured by the Svedberg unit (S). Prokaryotic ribosomes, found in organisms such as bacteria and archaea, are smaller, with a sedimentation rate of 70S. These 70S ribosomes consist of two subunits: a small 30S subunit and a large 50S subunit.
In contrast, eukaryotic ribosomes, found in organisms such as plants, animals, and fungi, are larger and have a sedimentation rate of 80S. Eukaryotic ribosomes are composed of a small 40S subunit and a large 60S subunit. The larger size of eukaryotic ribosomes reflects the increased complexity of eukaryotic cells and the more extensive array of proteins they produce.
These differences in ribosome structure have important implications, particularly in the development of antibiotics. Many antibiotics are designed to specifically target bacterial ribosomes (70S) without affecting the ribosomes of eukaryotic host cells (80S). This selective targeting allows for the treatment of bacterial infections with minimal impact on the patient’s own cells.
Where are ribosomes located within the cell?
Ribosomes can be found in two primary locations within the cell: free in the cytosol and bound to the rough endoplasmic reticulum (RER). Free ribosomes float freely within the cytosol and are responsible for synthesizing proteins that function within the cytosol itself or are transported to organelles such as the mitochondria, nucleus, or peroxisomes.
Bound ribosomes, on the other hand, are attached to the cytoplasmic surface of the rough endoplasmic reticulum (RER), giving the RER its characteristic “rough” appearance. These ribosomes synthesize proteins destined for secretion outside the cell, incorporation into the cell membrane, or use within lysosomes. After synthesis, these proteins are transported to the Golgi apparatus for further modification, sorting, and packaging.
The dual location of ribosomes reflects their versatile role in producing a wide range of proteins needed for various cellular functions, both within the cell and beyond.
How do ribosomes contribute to protein synthesis?
Ribosomes play a central role in protein synthesis, a process also known as translation. This process involves decoding the genetic information carried by messenger RNA (mRNA) into a sequence of amino acids, which are then linked together to form a protein. The sequence of amino acids in a protein determines its structure and function, making translation a critical step in gene expression.
Protein synthesis begins with the initiation phase, where the small ribosomal subunit binds to the mRNA and locates the start codon (AUG), which signals the beginning of the coding sequence. The large subunit then joins the small subunit to form a complete ribosome.
During the elongation phase, transfer RNA (tRNA) molecules bring specific amino acids to the ribosome, where they are added to the growing polypeptide chain. Each tRNA molecule has an anticodon that matches a codon on the mRNA, ensuring that the correct amino acid is added according to the genetic code.
The ribosome catalyzes the formation of peptide bonds between amino acids, linking them together to form a polypeptide chain. This process continues until the ribosome encounters a stop codon (UAA, UAG, or UGA), which signals the termination of translation. The newly synthesized protein is then released from the ribosome, ready to undergo folding and post-translational modifications before assuming its functional role within the cell.
What are ribosomopathies, and how do they relate to ribosome function?
Ribosomopathies are a group of disorders caused by defects in ribosome function or biogenesis. These disorders often result from mutations in genes encoding ribosomal proteins or other components involved in ribosome assembly and function. Ribosomopathies can lead to a wide range of health problems, including bone marrow failure, anemia, and congenital syndromes.
One example of ribosomopathy is Diamond-Blackfan anemia (DBA), a rare genetic disorder characterized by a failure of the bone marrow to produce sufficient red blood cells (RBCs). DBA is associated with mutations in genes encoding ribosomal proteins, leading to defective ribosome function and impaired red blood cell production. This results in anemia, a condition where the body does not have enough RBCs to carry oxygen to tissues.
Another example is Treacher-Collins syndrome (TCS), a congenital disorder that affects craniofacial development. TCS is caused by mutations in genes such as TCOF1, which encode proteins involved in ribosome biogenesis. These mutations disrupt ribosome function, particularly in neural crest cells, which are essential for craniofacial development. This leads to the characteristic facial abnormalities seen in TCS.
The study of ribosomopathies provides valuable insights into the fundamental processes of ribosome function and their impact on human health. Understanding these disorders can lead to the development of targeted therapies that address the underlying defects in ribosome biogenesis and function.
How do antibiotics target ribosomes, and why is this important in treating bacterial infections?
Antibiotics are a class of drugs designed to kill or inhibit the growth of bacteria, and many antibiotics achieve this by targeting bacterial ribosomes. The differences between prokaryotic ribosomes (70S) and eukaryotic ribosomes (80S) allow antibiotics to selectively target bacterial ribosomes without affecting the host’s ribosomes. This selective targeting is crucial for treating bacterial infections while minimizing harm to the patient’s cells.
For example, antibiotics such as
tetracyclines and aminoglycosides bind to the bacterial 30S subunit, interfering with the ability of the ribosome to accurately decode mRNA. This disruption prevents the synthesis of essential bacterial proteins, ultimately leading to bacterial death.
Other antibiotics, such as macrolides and chloramphenicol, target the bacterial 50S subunit, inhibiting the formation of peptide bonds and thus halting protein synthesis. By disrupting protein synthesis, these antibiotics effectively cripple the bacteria’s ability to grow, replicate, and survive.
The use of antibiotics to target bacterial ribosomes has been a cornerstone of modern medicine, revolutionizing the treatment of bacterial infections. However, the overuse and misuse of antibiotics have led to the emergence of antibiotic-resistant bacteria, posing a significant challenge to public health. Understanding the mechanisms by which antibiotics target ribosomes is essential for developing new strategies to combat antibiotic resistance.
What role do ribosomes play in genetic diseases?
Ribosomes play a crucial role in translating genetic information into proteins, and defects in ribosome function can lead to a range of genetic diseases. These diseases, collectively known as ribosomopathies, result from mutations in genes that encode ribosomal proteins or other components involved in ribosome biogenesis.
One example of a genetic disease linked to ribosome function is Diamond-Blackfan anemia (DBA), a rare disorder characterized by impaired red blood cell production. DBA is caused by mutations in genes encoding ribosomal proteins, leading to defective ribosome function and a decrease in the number of functional ribosomes within the cell. This disruption in ribosome biogenesis results in anemia and other health problems.
Another example is X-linked dyskeratosis congenita (DKC), a genetic disorder that affects multiple systems in the body, including the skin, nails, and bone marrow. DKC is caused by mutations in the DKC1 gene, which encodes a protein involved in ribosome biogenesis and telomere maintenance. The resulting defects in ribosome function contribute to the development of the disease.
These examples highlight the critical role that ribosomes play in maintaining cellular health and the severe consequences that can arise from ribosomal dysfunction. The study of ribosome-related genetic diseases provides valuable insights into the molecular mechanisms underlying these disorders and offers potential avenues for therapeutic intervention.
How do ribosomes differ from other cellular organelles?
Ribosomes differ from other cellular organelles in several key ways. Unlike membrane-bound organelles such as the nucleus, mitochondria, and endoplasmic reticulum, ribosomes are not enclosed by a membrane. Instead, they are composed of ribosomal RNA (rRNA) and proteins, forming two subunits that work together to carry out protein synthesis.
Another major difference is that ribosomes are responsible for translating genetic information into proteins, a process that is essential for virtually all cellular functions. This sets ribosomes apart from other organelles, which may be involved in specific cellular processes such as energy production (mitochondria), protein folding and modification (endoplasmic reticulum), or genetic material storage and processing (nucleus).
Ribosomes are also unique in their distribution within the cell. They can be found free-floating in the cytosol or attached to the rough endoplasmic reticulum (RER). This dual location allows ribosomes to produce a wide range of proteins, some of which are used within the cell, while others are destined for secretion or incorporation into cellular membranes.
Despite these differences, ribosomes work closely with other organelles to ensure the proper functioning of the cell. For example, after proteins are synthesized by ribosomes bound to the RER, they are transported to the Golgi apparatus for further processing and sorting before being sent to their final destinations.
What is the significance of ribosomal RNA (rRNA) in ribosome function?
Ribosomal RNA (rRNA) is a critical component of ribosomes and plays a central role in their function. rRNA is not only a structural element of ribosomes but also acts as a catalyst in the formation of peptide bonds during protein synthesis. This catalytic activity is essential for linking amino acids together to form proteins, making rRNA a key player in the translation process.
In the ribosome, rRNA molecules are organized into specific regions that contribute to the overall structure and function of the ribosome. For example, the 23S rRNA in the large subunit of prokaryotic ribosomes and the 28S rRNA in the large subunit of eukaryotic ribosomes are directly involved in the peptidyl transferase reaction, which forms peptide bonds between amino acids.
Additionally, rRNA helps maintain the proper alignment of mRNA and tRNA within the ribosome, ensuring that the genetic code is accurately translated into the corresponding protein sequence. The importance of rRNA in ribosome function is underscored by the fact that it is highly conserved across different species, indicating its fundamental role in cellular biology.
The discovery of rRNA’s catalytic activity challenged the long-held belief that only proteins could function as enzymes, leading to the concept of ribozymes—RNA molecules with enzymatic activity. This revelation has had profound implications for our understanding of the origins of life and the evolution of molecular biology.
What is the role of transfer RNA (tRNA) in protein synthesis?
Transfer RNA (tRNA) is a crucial molecule in the process of protein synthesis, acting as the adapter that translates the genetic code carried by messenger RNA (mRNA) into the corresponding sequence of amino acids. Each tRNA molecule carries a specific amino acid and has an anticodon that matches a complementary codon on the mRNA strand.
During translation, tRNA molecules enter the ribosome at the A site, where the anticodon of the tRNA pairs with the codon on the mRNA. This pairing ensures that the correct amino acid is added to the growing polypeptide chain according to the genetic instructions encoded in the mRNA.
Once the correct tRNA is in place, the ribosome catalyzes the formation of a peptide bond between the amino acid carried by the tRNA and the growing polypeptide chain. The tRNA then moves to the P site, where the polypeptide chain is transferred to the tRNA, and the process repeats with the next codon.
After the peptide bond is formed, the now-empty tRNA exits the ribosome through the E site, making room for a new tRNA molecule to enter the A site and continue the process. This cycle repeats until the ribosome encounters a stop codon, signaling the end of translation and the release of the newly synthesized protein.
The role of tRNA in protein synthesis is critical, as it ensures that the correct amino acids are added in the proper sequence, ultimately determining the structure and function of the resulting protein.
How do ribosomes interact with the endoplasmic reticulum (ER)?
Ribosomes interact with the endoplasmic reticulum (ER), specifically the rough endoplasmic reticulum (RER), to synthesize proteins destined for secretion, membrane incorporation, or use within organelles such as lysosomes. The RER is characterized by the presence of ribosomes attached to its cytoplasmic surface, giving it a “rough” appearance under a microscope.
When a ribosome begins translating an mRNA that encodes a protein with a signal peptide, the signal peptide directs the ribosome to the RER. The ribosome then becomes bound to the RER, and the growing polypeptide chain is translocated into the lumen of the RER through a channel called the translocon.
Once inside the RER lumen, the nascent protein undergoes folding and post-translational modifications, such as glycosylation, which are essential for its proper function. The RER also plays a role in quality control, ensuring that only properly folded and functional proteins are transported to the next stage of the secretory pathway.
After synthesis and initial processing in the RER, proteins are packaged into vesicles and transported to the Golgi apparatus for further modification, sorting, and packaging. From the Golgi, proteins are directed to their final destinations, whether it be the cell membrane, secretion outside the cell, or incorporation into organelles.
The interaction between ribosomes and the RER is essential for the production of proteins that are integral to various cellular processes, including cell signaling, membrane transport, and immune responses.
What happens when ribosomes malfunction, and what are the consequences?
When ribosomes malfunction, the consequences can be severe, leading to a range of disorders collectively known as ribosomopathies. These disorders arise from defects in ribosome biogenesis, structure, or function, often due to mutations in genes encoding ribosomal proteins or other components involved in ribosome assembly.
One of the most well-known ribosomopathies is Diamond-Blackfan anemia (DBA), a genetic disorder characterized by impaired red blood cell production. In DBA, mutations in ribosomal protein genes lead to defective ribosome function, resulting in a reduced number of functional ribosomes and subsequent anemia. Patients with DBA may also experience growth retardation, craniofacial abnormalities, and an increased risk of cancer.
Another example is Treacher-Collins syndrome (TCS), a congenital disorder that affects craniofacial development. TCS is caused by mutations in genes involved in ribosome biogenesis, leading to defective ribosome function in neural crest cells, which are crucial for craniofacial development. The result is a range of facial abnormalities, hearing loss, and other developmental issues.
Ribosome malfunctions can also lead to broader systemic effects, as ribosomes are essential for the production of all cellular proteins. A
disruption in protein synthesis can impair cell growth, division, and stress response, ultimately leading to cell death. This underscores the importance of ribosomes in maintaining cellular health and highlights the potential for therapeutic interventions targeting ribosome function in the treatment of ribosomopathies and other related disorders.
How do ribosomes evolve, and what does this tell us about the origins of life?
Ribosomes are among the most ancient and conserved structures in all living organisms, reflecting their fundamental role in cellular biology. The evolution of ribosomes is closely tied to the origins of life, as they are essential for the translation of genetic information into functional proteins.
The study of ribosome evolution has revealed that ribosomes have a highly conserved core structure across all domains of life—Bacteria, Archaea, and Eukarya—suggesting that the last universal common ancestor (LUCA) possessed a ribosome similar to those found in modern cells. This conserved structure, primarily composed of ribosomal RNA (rRNA), is thought to have originated in the RNA world, a hypothetical early stage of life where RNA served both as genetic material and as a catalyst for biochemical reactions.
The catalytic activity of rRNA, particularly in the formation of peptide bonds, supports the idea that early ribosomes were primarily composed of RNA, with proteins being added later in evolution to enhance ribosome function and stability. This RNA-centric view of ribosome evolution provides insights into the transition from an RNA world to a more complex, protein-based world.
The evolutionary history of ribosomes also sheds light on the origins of life itself. The ability of ribosomes to translate genetic information into proteins is a critical step in the development of living organisms, and the conservation of ribosome structure across all life forms suggests that this process was established very early in the history of life.
Understanding ribosome evolution not only informs our knowledge of the origins of life but also has practical implications for fields such as synthetic biology, where researchers are exploring the possibility of creating artificial ribosomes or modifying existing ones to produce novel proteins with therapeutic or industrial applications.
Read More Articles
- The Five Kingdom Classification With Perfect Examples
- Eubacteria: Structure, Characteristics, Classification, And Types
- Archaebacteria: A Glimpse Into Ancient Life Forms
- Kingdom Protista: A Diverse Group Of Single-Celled Eukaryotes
- The Diversity And Importance Of Bacteria: Archaebacteria And Eubacteria
- Kingdom Monera: The World Of Bacteria